|
Tribological performance of PFPE and X-1P lubricants at head-disk interface. Part II. Mechanisms
Zheming Zhaoa, Bharat Bhushana and Czeslaw Kajdasb
a Computer Microtribology and Contamination Laboratory, Department of Mechanical Engineering, The Ohio State University, Columbus, OH 43210-1107, USA
b Warsaw University of Technology, Institute of Chemistry, Plock, Poland
This paper, with the concepts of hydrogen bonding interaction and tribo-emission, develops a new approach of the mechanism of perfluoropolyether (PFPE) lubricant degradation at the head-disk interface. The role of lubricant X-1 P in tribological performance is also described. The mechanism is as follows: (1) at the interface, there exist hydrogen atoms with partial positive charge and oxygen atoms with partial negative charge; (2) hydrogen bonding interactions at the sliding interface result in high friction which depletes the lubricant film at some sites; (3) low energy electrons are emitted from the sites with solid-solid asperity contact, inducing C—C bond scission through the interaction of low-energy electrons with PFPE lubricant molecules. Carbon overcoat on Al2O3-TiC surface passivates the interaction between water and PFPE lubricant molecules. Hydrogen bonding interactions are minimized during the presence of lubricant X-1P. The new approach well explains experimental results in part I of the paper.
Keywords: lubricant, friction, wear, degradation mechanism, thin-film disks
* — Published in : Tribology Letters Vol., 6, (1999) 141-148
1. Introduction
Perfluoropolyether (PFPE) lubricants, such as Fomblin Z-Dol and Demnum SA, are popularly used in rigid disk drives [1]. However, one of the major tribological problems at the head-disk interface is the decomposition of PFPE lubricants, resulting in wear of the carbon overcoat consequently head-disk interface failure. Advanced lubricants for disk drive applications are continually developed for high-density recording, proximity recording and even contact recording. Lubricant X-1P is recently used as an additive to PFPEs in disk drives. Fomblin Z-type and Demnum SA lubricants are a random copolymer of CF2CF2O and CF2O with a linear structure whereas X-1P is a mixture of p-fluorophenoxy- and m-trifluoromethylphenoxy-substituted cyclic phosphazenes, with a spherical structure; it contains four m-trifluoromethylphenoxy and two p-fluoro-phenoxy substituted groups (table 1). It is known that PFPE lubricants are more likely to be degraded at wet environmental condition if alumina is used as a mating material whereas lubricant X-1P is much less degraded. Understanding of the PFPE lubricant decomposition and X-1P's role is required to meet the need of the continuously increased areal density in magnetic recording.
Lubricants with polar end groups, known as functional or polar lubricants, are designed to provide enhanced adhesion to the diamond-like carbon (DLC) overcoats. Groups such as hydroxyl (—OH), carboxyl (—COOH), or piperonyl (—CH2—phe═(O)2═CH2) can react with surfaces and bond the lubricant to the DLC surface. These lubricants form bonded lubricant films and are effective in reducing friction and increasing durability [2-4]. Examples of non-polar and polar molecules are shown in table 1. In the case of Z-Dol, a hydrogen atom, covalently bonded with an oxygen atom in the O—H bond, has a partial positive charge on the end of the bond. This hydrogen atom can be easily attracted to the negative charge of other molecules. Likewise, the lone pairs of electrons in the oxygen atom can be attracted to positive charges of other molecules. Consequently, a dipole moment develops in such a way that one portion is negatively charged while another portion is positively charged. Hydroxyl group —OH, as a part of an alcohol group in Z-Dol molecule or water molecule structure, can form hydrogen bonds through its hydrogen atom (H) and the unshared pairs of electrons on the oxygen atoms (O) of neighboring molecules, resulting in an intrinsically polar molecule. Hydrogen bonds are quite strong, on the order of 10-40 kJ/mol. Water molecule, as an example of hydrogen bonds, has the adequate balance of hydrogen atoms and electron pairs to form the maximum number of hydrogen bonds:
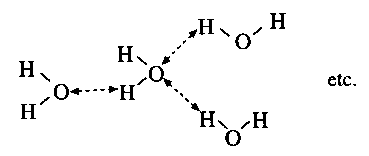 | etc |
Thus, the interaction between polar lubricant atoms (H and O, as in Z-Dol) and/or surface hydroxyl groups can be very significant. In Z-15, even though the lone pairs of electrons in the fluorine atom are unshielded and thus exhibit electronegativity, the —CF3 end is symmetric so its polarity is low and negligible as compared to the polarity of the —OH end. Also, X-1P is a non-polar lubricant.
Table 1.
Chemical structure and selected properties of perfluoropolyether lubricant Z-15, Z-Dol and SA, and lubricant X-1P. |
Lubricant | Formula | End Groups | Molecular Weight (Daltons) | Density (x10³ kg/m³ at 20°C) | Kinematic viscosity (mm²/s) |
Z-15 | CF3—O—(CF2—CF2—O)m—(CF2—O)n—CF3 | —CF3 | 9100 | 1.84 | 150 @ 20°C |
Z-DOLa | HO—CH2—CF2—O—(CF2—CF2—O)m—(CF2—O)n—CF2—CH2—OH (m/n ~ (2/3) | —OH | 2000 | 1.81 | 80 at 20°C 34 at 40°C |
SAa | F—(CF2—CF2—CF2—O)m—CF2—CF2—CH2—OH | —OH | 3600 | 1.87 | 75 @ 40°C |
X-1P | 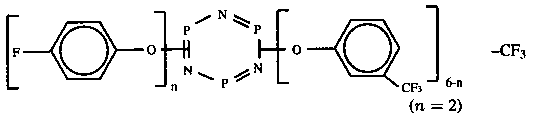 | —CF3 | 1000 | 1.48 | 1826 @ 20°C 205 @ 40°C |
a — Functional or polar lubricants
|
In this paper, focus is given to the interface of head slider (Al2O3—TiC or Al2O3—TiC with DLC overcoat) and disk with amorphous-hydrogenated carbon (a:C—Hx) overcoat. A systematic approach of the mechanism of PFPE lubricant degradation is developed to understand how lubricants are degraded and the role of lubricant X-1P. This approach well explains the experimental results of lubricant X-1P and Z-Dol presented and discussed in part I [5].
2. Importance of low-energy electrons in the PFPE lubricant degradation process
2.1. A survey of degradation mechanisms
There are four mechanisms considered to decompose the PFPE molecules: (1) thermal decomposition reactions by frictional heating; (2) catalytic reactions by materials of the head sliders or disks; (3) the scission of the PFPE molecules by mechanical shearing, and (4) triboelectrical reactions by electrons emitted from the wearing solid surfaces. Thermal decomposition temperature of the PFPE lubricants has been reported to be above 350 °C [6] which can be reached by the transient temperature on microsecond scale [1,7]. The decomposition can occur by oxidation and chemical breakdown at high interface temperatures. Kasai et al. [8] and
Kasai [9] reported that Lewis acid sites present on Al2O3 are catalytic and cause PFPE lubricant molecules decomposition. Lewis acid is defined as a substance that can accept a pair of electrons. Their experiments had been conducted with ground-up powders of Al2O3 in the presence of lubricant at high temperatures ranging from 200 to 300 °C for a duration of 1-48 h. Kinetics of these experiments is very different from that in the drive operation. Novotny et al. [10] anticipated that PFPE lubricants are decomposed by the scission of the polymer chains, driven by several processes - high shear rates, high interfacial temperature and tribocharging. Perettie et al. [11] conjectured that there was no decomposition of X-1P in contact with the Al2O3—TiC surface although no data were presented to support their evidence. Vurens et al. [12] reported the evidence that decomposition of PFPEs during wear was an electron-mediated process. In their analytical investigation into the degraded lubricant X-1P, Keller and Saba [13] reported that oligomerization to be the dominant mode of degradation, resulting in most of the observed viscosity increases. Their GC/MS, FT-IR, and P NMR data confirmed the existence of a cyclotetramer structure in the degraded fluid.
2.2. Tribo-emission
The tribo-emission may be a significant factor in tribochemical reactions in the contact zone (operating under lightly loaded conditions) in addition to temperature rise and the catalytic action of the sliding surface. It is known that a wide variety of materials after mechanical treatment become electron emitters. The term "exoelectron emission" originates from investigation of the emission in freshly formed metals which were accounted for as a consequence of exothermal transformation process of the surface [14]. As reported in the literature [15-19], exoelectron emission occurs when a material's surface is disturbed by plastic deformation, abrasion, fatigue cracking or phase transformation. The electron emission from a freshly formed surface reaches a maximum almost immediately then decays with time [15,20]. Electron emission has been observed from both metals and nonmetals. The energy of exoelectrons is considered to be very low, of the order of 2-3 eV [21]. At present, the term exoelectron emission is used as an
inclusive term for emission phenomena which proceed unsteadily, with changed work function, and after excitation by non-thermal energy. Recently, Tagawa et al. [22] investigated the tribo- and photo-stimulated exoelectron emission from reactor graphite disk against pin (material SUS304). They found that the variation of tribo-stimulated exoelectron emission from graphite corresponded well with the variation of the friction coefficient. Figure 1 shows the changes in the emission intensity from a graphite disk and the friction coefficient over 0-4 revolutions of sliding in a pin-on-disk tester.
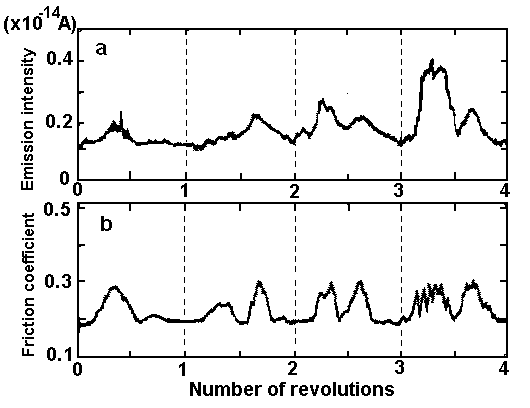 |
Figure 1. Variations of
(a) tribo-stimulated exoelectron emission and
(b) friction coefficient, obtained from reactor graphite sliding against SUS304 pin in a pin-on-disk test [22].
|
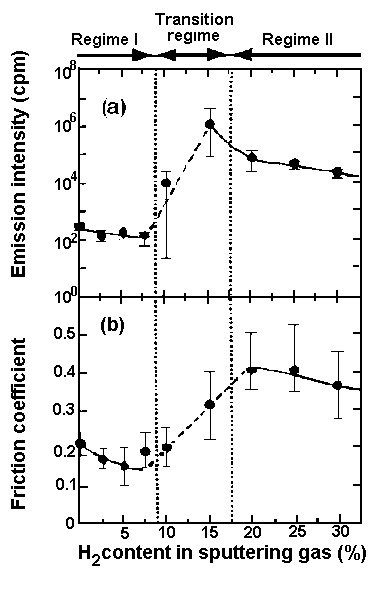 |
Figure 2.
- Dependence of electron emission intensity and
- friction coefficient on H2 content in sputtering gas,
during wear of carbon films sliding against alumina in a ball-on-disk test [23].
|
Nakayama et al. [23] investigated the friction, wear and triboelectron emission of alumina sliding on a:C—Hx films in ambient air, and established that carbon films prepared with low level hydrogen gas contents showed low friction and low wear in the production of low electron emissions.
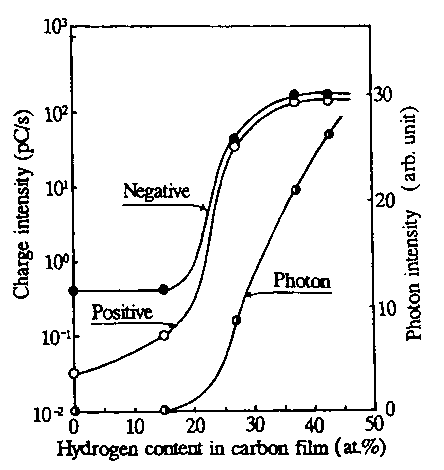 |
Figure 3. Relation between charge intensity accumulated for 1 s and hydrogen content in carbon film sliding against diamond stylus in a pin on-disk test [24].
|
With intermediate level hydrogen gas content, transitions of friction, wear intensity and electron emissions occurred from low to high. At high hydrogen gas content, high friction and high wear occurred to produce high electron emissions. Figure 2 shows the dependence of the triboemission intensity of electrons in counts/min and the friction coefficient on hydrogen gas content in the sputtering gas in the sliding test of alumina sliding on amorphous-hydrogenated carbon (a:C) and a:C-Hx; films. Most recently, Nakayama et al. [24] investigated tribo-emission of negatively charged particles and positively charged particles, tribocharging and the friction coefficient in a frictional system with diamond sliding on hydrogenated carbon films in ambient air. They found that in the region of low hydrogen content, at and below 15 at%, both negatively and positively charged particles were emitted, but no photon emission was observed. In the region with hydrogen contents between 15 at% and 37 at%, emission intensity of the charged particles increased sharply, accompanied by tribo-photons. Figure 3 clearly depicts this situation and shows that with a further increase in hydrogen content, the emission intensity of the negatively and positively charged particles and photons increased. Interestingly, the friction coefficient also increased from a low to a high value in the regime of hydrogen content between 15 at% and 27 at%, so that the regime of charge transition and friction transition do not correspond [24]. The attachment of the emitted electrons degrades lubricant molecules through C—O bonding scission in Z-Dol.
2.3. Negative-ion-radical action mechanisms
According to the negative-ion-radical action mechanism (NIRAM) [25,26] tribochemical reactions can be initiated by low-energy electrons (exoelectrons) spontaneously emitted from most solid nascent surfaces formed during friction. The concept is based upon the ionization mechanism of lubricant component molecules caused by the action of electrons of low energy (1-4 eV). The principal thesis of the model is that lubricant components, for example, alcohols [25], form anions which are then chemisorbed on the positively charged areas of rubbing surfaces. Recently, Vurens et al. [27] examined the bonding of the thin PFPE films by low-energy electrons and UV. They showed that the bonding takes place through the interaction of the PFPE molecules, with a low-energy photoelectron emitted by excitation of the substrate by the UV photons. Vurens et al. [12] have also shown that for a Demnum S200 film on a diamond-like carbon substrate bombarded with low-energy electrons, the most abundant fragment ions comprise the following negative species: F¯, C3F5O2¯ and C3F7O2¯. Thus the electron mediated degradation mechanism of PFPE lubricants seems to be of particular importance.
3. Interaction details on the head-disk interface
3.1. Role of adsorbed water film
Oxide surfaces, unless highly dried, are usually covered with hydroxyl groups formed by chemisorption of water [28]. Various chemical distinct types of hydroxyl groups also persist on the surface of alumina even after drying at 400-500 °C [29]. Removal of the groups from alumina leaves a strained surface on which strained oxide linkages have been postulated as active sites [30].
Alumina in a form is used in head sliders. One study of adsorption on a-alumina was that of Carruthers et al. [31], who determined surface area and porosity using nitrogen and argon adsorption. The authors concluded that the high affinity exhibited by a-alumina for water is associated with a marked tendency for exposed aluminum ions to be rehydrated, thus allowing dissociative chemisorption of water to precede at low relative pressure (p>/po), and followed by specific physical adsorption. Bailey and Wightman [32] reported that a-alumina adsorbs more water vapor per unit area than g-alumina.
Figure 4(a) shows a schematic of water interaction on the Al2O3-TiC surface. Hydroxyl (—OH) groups are chemisorbed from environment onto the Al2O3-TiC surface. Under the humid environmental condition, an adsorbed thin water film will interact with the chemisorbed —OH groups through partially negatively charged oxygen atom and partially positively charged hydrogen atom. The higher the humidity, the more interacted water molecules are present. In the case of Al2O3-TiC surface with DLC overcoat, figure 4(b), instead of hydroxyl —OH, carbon functional groups
are the most chemisorbed groups to the surface. The water molecules interact through hydrogen bonding with these functional groups, forming more strongly adsorbed film. Dangling bonds may also play a part in better water molecule adsorption to the carbon overcoat [33].
• Danglig bonds; — Covalent bond, ↔ Hydrogen bond interaction
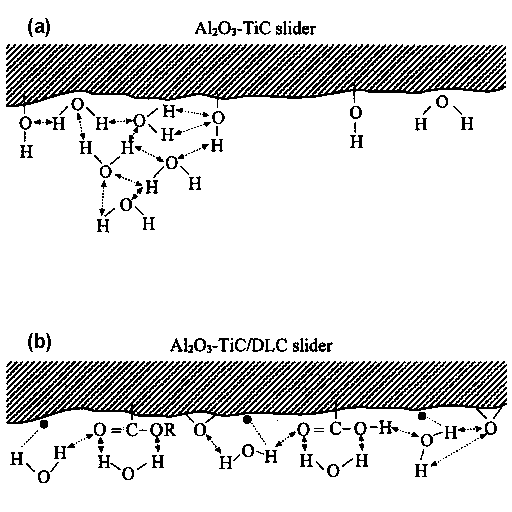 |
Figure 4. Schematic of water film interaction on
- Al2O3-TiC and
- Al2O3-TiC/DLC surfaces.
|
One of conceivable interaction between water molecules and the DLC atom can proceed through the water hydrogen atom and the surface dangling bond. Dangling bonds are free radicals present in DLC overcoats. A free radical is an atom or group of atoms having one or more unpaired electrons. It is symbolized with a single dot representing the unpaired electron, for example: F•; HO•; CF3•; CF3—O•; CF3—CF2• etc. They have no positive or negative charge, however, they are very reactive. A free radical is highly reactive intermediate because of its unpaired electron.
To describe the interaction between lubricant and water molecules, table 2 shows hydrophile-lipophile balance (HLB) number for selected groups [34,35]. The HLB number gives a quantitative way of correlating the chemical structure of surfactant molecules with their surface activity. The hydroxyl (—OH) group and oxygen (—O—) group favor water adsorption so they belong to the hydrophilic while —CF3 group is in the hydrophobic category. Among the lubricants listed in table 1, Z-Dol is with —OH groups at both ends while Demnum SA is with only one —OH end group. Furthermore, backbone of Z-Dol is with higher frequency of oxygen linkage in the ether group (number of oxygen atoms per CF2 molecules) than Demnum SA. It is expected that Z-Dol would be more favorable to water molecules. The test results of static friction, kinetic friction and durability have confirmed that lubricant Demnum SA is relatively insensitive to humid environment as compared to that of lubricant Z-Dol [3]. Lubricant X-1P involves no hydrophilic end groups. There is no interaction between its end groups and water molecules. The structure of X-1P makes no hydrogen bonding interactions as existed in PFPE lubricants available at the interface.
Table 2.
Hydrophile-lipophile balance (HLB) number for selected groups. |
Group | HLB number |
Hydrophilic |
—COOR | 2.4 |
—COOH | 2.1 |
—OH | 1.9 |
—O— | 1.3 |
Hydrophobic |
—CH2— | -0.475 |
—CH3 | -0.475 |
═CH— | -0.475 |
—CF2— | -0.87 |
—CF3 | -0.87 |
Miscellaneous |
—(CH2CH2O)— | 0.33 |
—(CH2CH2CH2O)— | -0.15
|
3.2. Tribo-emission at the head-disk interface
There is no reference directly referred to tribo-emission of the head-disk interface. However, Nakayama et al. [23] simulated the head-disk interface using the friction system of alumina sliding on a:C—Hx; films and found that both negatively charged particles and positively charged particles are emitted under friction in ambient air.
3.3. Lubricant X-1P and Z-Dol on DLC overcoat
When lubricant X-1P and Z-Dol are applied on disks with DLC overcoat, they behave differently. Lubricant X-1P is non-polar so it can not be bonded to DLC layer. The phosphazene ring in X-1P may exist in a puckered form or planar form [36]. Figure 5 shows the structure of planar phosphazene ring and puckered phosphazene ring of X-1 P by the molecular mechanics method [37]. In the planar form, the phosphazene ring is sterically shielded by the six phenoxy groups, and it is unlikely that strong adhesive interaction occurs between partially fluorinated phenyl rings and the carbon overcoat. In the puckered form, a basal plane is defined by the nitrogen atoms and three phenoxy groups in the equatorial positions, and the three phenoxy groups in the axial positions are directed upward. A strong interaction is then possible between the exposed phosphazene ring and the carbon overcoat. With X-1P molecules adsorbed in this orientation, partially fluorinated phenyl groups projected upward would account for the good lubricity shown by disks lubricated with mono layer level of neat X-1P [5,11]. It can be speculated that X-1P exists in the form of puckered ring and adsorbs strongly onto the carbon overcoat of thin film disks.
Lubricant Z-Dol is polar so it can be bonded to the DLC overcoat. Only a small amount of the functional groups, carboxyl and hydroxyl present on the carbon surface show strong affinity to the lubricant. Main adsorption sites are associated with the presence of unpaired electrons (dangling bonds). Figure 6 shows the schematic for the adsorption of Z-Dol molecule on the carbon surface
at 20 °C [33]. C—O bond and the hydroxyl groups in the case of Z-Dol interact with functional groups and dangling bonds on the carbon surface. The functional groups behave as an anchor, i.e., immobile fraction of lubricant film. The addition of an alcohol (—OH) end group to a lubricant molecule adds on the order of 20 kJ/mol interaction energy even if no chemical reaction occurs with the surface.
3.4. PFPE lubricant C—O bond scission
Comparing bond dissociation energies of the PFPE lubricant bonds, including the polar lubricant Z-Dol, one can see
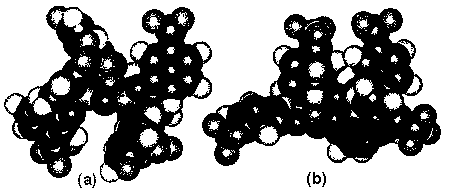 |
Figure 5. Structure of (a) planar phosphazene ring and (b) puckered phosphazene ring of X-1P by the molecular mechanics method [37].
|
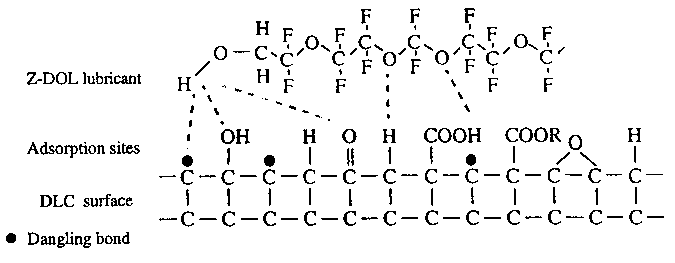 |
Figure 6. Schematic for the Z-DOL molecules adsorbed on the DLC surface at 20 °C.
|
Table 3.
Primary bond energies of various bonds in PFPE lubricants. |
Bond | Dissociation energy (kJ/mol) |
C—F | 473 |
O—H | 464 |
C—H | 414 |
C—O | 360 |
C—C | 347
|
from table 3 that the C—C bond is the least stable one. On the other hand, however, Matsunuma et al. [38], using semiempirical molecular orbital (MO) simulation, showed that electron attachment to a PFPE model (referred to as PMO) having a methylene oxide segment reduced the bond order of the C—O bond. Accordingly, it is plausible to assume that lubricant C—O bond scission is induced through the interaction of low-energy electrons with PFPE lubricant molecules. Cleavage of the weakest C—O bond of the formed anion-radical produced anion and free radicals. This is in line with the NIRAM approach [25,26]. Summarizing the results obtained by Matsunuma et al. [38] it is reasonable to suggest that ionic intermediates play an important role in electron-induced degradation of PFPE lubricants.
4. Proposed mechanism of PFPE degradation and experimental evidence
For Al2O3-TiC head slider sliding against disk lubricated with Z-Dol at high humidity, the following steps of a degradation mechanism are proposed:
- The adsorption process of water molecules is controlled by the surface property. Alumina surface, unless highly dried, contains hydroxyl groups. DLC overcoats include a variety of functional groups.
- Molecules of PFPE lubricants such as Z-Dol are characteristic of partial positive charge on hydrogen and partial negative charge on oxygen.
- Hydrogen bonding interactions at sliding surfaces result in higher friction. The high friction at the interface depletes lubricant film at some sites where solid-solid asperity contact will occur.
- Low energy (1-4 eV) electrons are thus emitted from the sliding surfaces where solid-solid asperity contacts occur. Emitted electrons interact with lubricant molecules.
- Lubricant C—O bond scission is induced through the interaction of low-energy electrons with PFPE lubricant molecules. Lubricant molecules are thus decomposed.
We now present discussion on experimental results in the part I of the paper [5] using the mechanism just mentioned. A schematic of head-disk interaction via hydrogen bonding is shown in figure 7(a). The head slider is made of Al2O3-TiC, and the disk is with amorphous-hydrogenated carbon (a:C—Hx) overcoat and lubricated with lubricant Z-Dol. On the disk surface there are some functional groups ( —COOR, —OOH, —OH) that also interact with the bonded Z-Dol molecules (figure 6). The head-disk interaction strength is controlled by the water film thickness on the Al2O3-TiC surface. The thicker the adsorbed water film, the closer
contact between the head and disk, resulting in the increase of the interaction strength. Water molecules are also adsorbed to the disk surface. The higher the humidity, the thicker water film is formed. Some micro water droplets can penetrate the lubricant film, displacing it from the surface. The upward side of Z-Dol film, particularly at the —CF2—CF2—OH end, will interact stronger with the Al2O3-TiC layer.
In the case of head Al2O3-TiC with carbon overcoat (figure 7(b)), the water molecules interact with the carbon overcoat functional groups of head surface. Dangling bonds may also play a part in better water molecule adsorption to the carbon overcoat. One of conceivable interaction between water molecules and the DLC atom can proceed through the water hydrogen atom and the surface dangling bond. As the consequence the hydrogen bonding interaction between the head and disk is significantly reduced in comparison with figure 7(a). Lower hydrogen bonding interaction prevents from high friction at the interface. Lubricant film continually covers the surfaces and an increase in the tribo-emission during sliding through solid-solid asperity contact is alleviated. Accordingly, lack of tribo-emission postpones the degradation of PFPE lubricant molecules.
In part I [5] it is shown that, lubricant Z-Dol provides poor durability in drag, CSS and ball-on-flat tests at high humidity (80%) compared to X-1P.
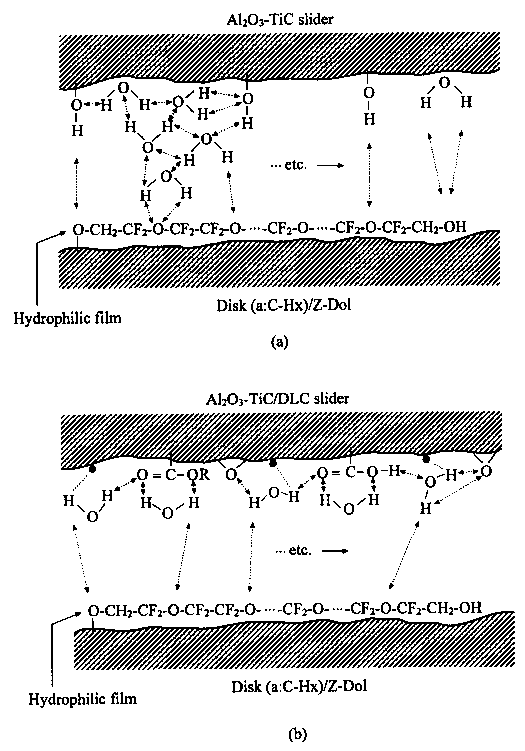 |
Figure 7. Schematic of
- Al2O3-TiC and
- Al2O3-TiC/DLC and
(a:C—Hx) disk/Z-Dol interaction via hydrogen bonding.
|
It is the stronger hydrogen bonding interaction which causes high friction followed by depletion of lubricant film at some sites on the lubricated disk surface. The solid-solid asperity contact at the sliding interface emits the low voltage electrons. The attachment of the emitted electrons degrades lubricant molecules through C—O bonding scission in Z-Dol. Consequently, more solid-solid asperity contact forms followed by more electrons being emitted on the sliding surface. Such a cycle continues until the lubricant film is completely degraded. In the case of DLC coated Al2O3-TiC slider, the carbon coating on the slider surface confines the water molecules and minimizes strong interactions of hydrogen bonding at the interface. The lack of hydrogen bonding interaction keeps friction low and avoids more electrons being emitted from solid-solid contact. Lubricant degradation is postponed.
When lubricant X-1P is applied on the disk surface instead of lubricant Z-Dol, as shown in figure 8(a), there is no hydrogen bonding between the two surfaces. Hydrophobic —CF3 and —F layer repels water film from itself. In the case that the disk surface totally covered by X-1P molecules, water molecules present on the covered surface will be confined between the surface and the P— ring hydrophobic substituents.
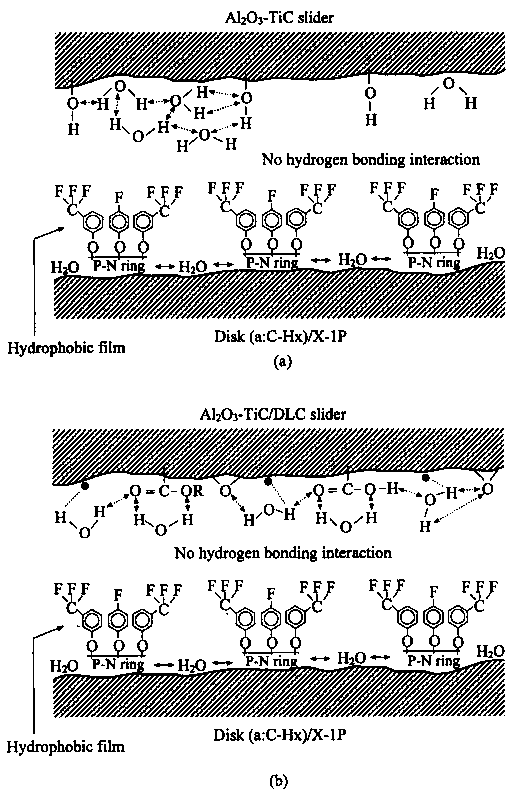 |
Figure 8. Schematic of
- Al2O3-TiC and
- Al2O3-TiC/DLC and
(a:C—Hx) disk/Z-Dol interaction via hydrogen bonding.
|
Some water molecules might be confined within
|
groups which can form hydrogen bonding. In the case of the disk surface with some sites not covered by X-1P molecules, water molecules will be adsorbed on the a:C—Hx surface sites and therefore they will be far away from the adsorbed water molecules on the surface of Al2O3-TiC slider. Without the hydrogen bonding interaction, the coefficient of friction remains low. On the other hand, compared to Z-Dol, X-1P's higher viscosity and its spherical molecule structure will provide stronger lubricant film in preventing directly solid-solid asperity contact at the interface. This is confirmed by Yang and Chung [39] that in their pin-on-disk test, the sliding interface provides much higher contact resistance in the case of using lubricant X-1P. Therefore, none of hydrogen bonding interactions results in low friction at the interface; lubricant film will not be depleted so the number of solid-solid asperity contact sites do not increase; the density of low-energy electrons emitted at the sliding interface will not increase. It is the reason why lubricant X-1P is not degraded in drag, CSS and ball-on-flat sliding tests.
In the case of Al2O3-TiC TDLC and disk (a:C—Hx)/X-1P system, water molecules are better confined on the head slider surface (figure 8(b)). Because no hydrogen bonding interaction forms at the interface as shown in figure 8(a), the role of carbon in confining water molecules is not so critical to tribological performance as that in the case of lubricant Z-Dol. Consequently, similar tribological performance is obtained from CSS tests between Al2O3-TiC slider with and without DLC overcoat at high humidity level [5].
5. Conclusions
The role of lubricant X-1P in tribological performance is described and compared with lubricant Z-Dol. A new mechanism approach of PFPE lubricant degradation is de veloped, specially for the Al2O3-TiC surface sliding against disk with lubricant Z-Dol at high environmental humidity. The hydrogen bonding interaction, between water molecules and lubricant molecules through partially positively charged —H atoms and partially negatively charged —O— atoms, results in high friction at the interface and causes solid-solid asperity contact at some sites from where low-energy electrons are emitted. Lubricant molecules are further degraded by the attachment of emitted low-energy electron to C—O bonds and thus C—O bond scission. Carbon overcoat on the slider alleviates the interaction between water and lubricant molecules so that it postpones the PFPE lubricant degradation. There is no hydrogen bonding interaction between water molecules and lubricant X-1P. The mechanism explains well the degradation process of PFPE lubricants such as Z-Dol, and much less degradation of lubricant X-1P.
Acknowledgement
Financial support for this study was provided by the industrial membership of the Computer Microtribology and Contamination Laboratory (CMCL).
|
References
- [1]
- B. Bhushan, Tribology and Mechanics of Magnetic Storage Devices, 2nd Ed. (Springer, New York, 1996).
- [1]
- Z. Zhao and B. Bhushan, Wear 202 (1996) 50.
- [2]
- B. Bhushan and Z. Zhao, IEEE Trans. Magn. 33 (1997) 918.
- [3]
- B. Bhushan and Z. Zhao, J. Info. Storage Proc. Syst. 1 (1999) 1.
- [4]
- Z. Zhao and B. Bhushan, Tribol. Lett. 6 (1999) 129.
- [5]
- L.S. Helmick and W.R. Jones, TM-102493, NASA, 1990.
- [6]
- B. Bhushan, ASME J. Tribol. 114 (1992) 420.
- [7]
- P.H. Kasai, W.T. Tang and P. Wheeler, Appl. Surf. Sci. 51 (1991) 201,
- [8]
- P.H. Kasai, J. Info. Storage Proc. Syst. 1 (1999) in press.
- [9]
- V.J. Novotny, T.E. Karis and N.W. Johnson, ASME J. Tribol. 114 (1992) 61.
- [10]
- D.J. Perettie, W.D. Johnson, T.A. Morgen, K.K. Kar, G.E. Potter, B.M. DeKoven and C.L. Putzig, Adv. Info. Storage Syst. 7 (1996) 157.
- [11]
- G.H. Vurens, R. Zehringer and D. Saperstein, in: Surface Science Investigations in Tribology, Vol. 485, eds. Y.W. Chung, A.M. Homola and G.B. Street (American Chemical Society, Washington, DC, 1992) ch. 9, pp. 169-180.
- [12]
- M.A. Keller and C.S. Saba, Analytical Chemistry 68 (1996) 3489.
- [13]
- J. Kramer, Der Metallische Zustand (Vandenhoek and Ruprecht, Goettingen, Germany, 1950).
- [14]
- L. Grunberg, Brit. J. Appl. Phys. 9 (1958) 85.
- [15]
- F. Brotzen, Phys. Stat. Sol. 22 (1967) 9.
- [16]
- J. Moore and S. Tsang, in: STP 515 (ASTM, Philadelphia, PA, 1972) pp. 113-117.
- [17]
- W. Baxter, Vacuum 22 (1974) 571.
- [18]
- P.A. March and E. Rabinowicz, ASLE Trans. 20 (1977) 315.
- [19]
- C. Veerman, Materials Sci. and Eng. 4 (1969) 329.
- [20]
- S.W. Chaikin, Wear 10 (1967) 49.
- [21]
- M. Tagawa, M. Mori, N. Ohmae, M. Umeno and S. Takenobu, Tribol. International 26 (1993) 57.
- [22]
- K. Nakayama, K. Yamanaka, H. Ikeda and T. Sato, Tribol. Trans. 40 (1997) 507.
- [23]
- K. Nakayama, B. Bou-said and H. Ikeda, ASME J. Tribol. 119 (1997) 764.
- [24]
- C. Kajdas, Wear 116 (1987) 167.
- [25]
- C. Kajdas, Lubrication Science 6 (1994) 203.
- [26]
- G.H. Vurens, C.S. Gudeman, L.J. Lin and J.S. Foster, Langmuir 8 (1992) 1165.
- [27]
- J.B. Peri, J. Phys. Chem. 69 (1965) 220.
- [28]
- J.B. Peri, J. Phys. Chem. 69 (1965) 211.
- [29]
- E.B. Cornelius, T.H. Milliken, G.A. Mills and A.G. Oblad, J. Phys. Chem. 59 (1955) 809.
- [31]
- J.D. Carruthers, D.A. Payne, K.S.W. Sing and L.J. Stryker, J. Colloid and Interface Sci. 36 (1971) 205.
- [32]
- R.R. Bailey and J.P. Wightman, J. Colloid and Interface Sci. 70 (1979) 112.
- [33]
- M. Yanagisawa, in: Tribology and Mechanics of Magnetic Storage Systems. SP-36, Vol. 9 (STLE, Park Ridge, IL, 1994) pp. 25-32.
- [34]
- J.T. Davies and E.K. Rideal, Interfacial Phenomena, 2nd Ed. (Academic Press, London, 1963).
- [35]
- D. Myers, Surfaces, Interfaces and Colloids: Principles and Applications (VCH Publishers, New York, 1991).
- [36]
- H.R. Allcock, Phosphorus-Nitrogen Compounds: Cyclic, Linear, and High Polymeric Systems (Academic Press, New York, 1972).
- [37]
- N.L. Allinger, Adv. in Phys. Org, Chem. 13 (1976) 1.
- [38]
- S. Matsunuma, T. Miura and H. Kataoka, Tribol. Trans. 39 (1996) 380.
- [39]
- Z. Yang and Y.W. Chung, Tribol. Trans. 39 (1996) 974.
| |